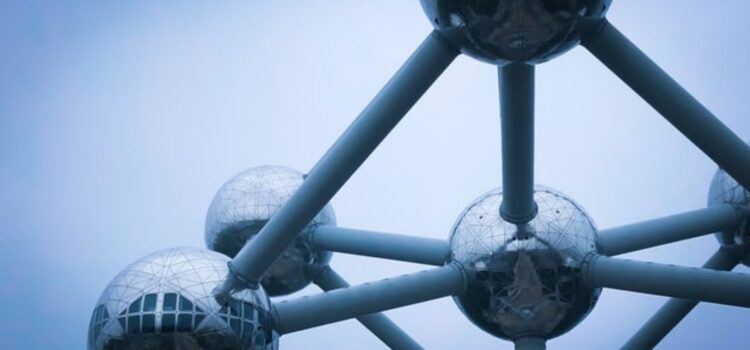
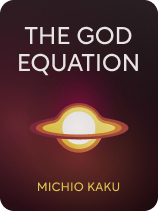
This article is an excerpt from the Shortform book guide to "The God Equation" by Michio Kaku. Shortform has the world's best summaries and analyses of books you should be reading.
Like this article? Sign up for a free trial here.
What is the Standard Model of physics? How close is it to a unified theory? What’s it missing?
Scientists have yet to figure out the unifying theory—what theoretical physicist Michio Kaku calls “the God Equation.” But, physicists have made a great deal of progress using an intermediate step toward that theory. They refer to it as the Standard Model.
Keep reading to understand the Standard Model, what it tells us about the universe, and what it doesn’t explain.
The Standard Model of Physics
We’ll go over the basis of the Standard Model as well as its theoretical problems, including cumbersome mathematics, a mismatch between the theory and the observable universe, and its current inability to account for the force of gravity.
So, what is the Standard Model of physics? Beginning in the 1970s, physicists stitched together the equations for the strong nuclear force, the weak nuclear force, and electromagnetism to produce what’s now called the Standard Model. This mathematical expression of those fundamental forces presumes that, in the extreme conditions that existed moments after the Big Bang, all of the forces acted as one and diverged only as the universe cooled.
(Shortform note: In Astrophysics for People in a Hurry, Neil deGrasse Tyson goes into more detail about the universe’s first moments and their importance to a unified theory of physics. Tyson explains that at the instant of the universe’s inception, the domains of relativity and quantum mechanics overlapped. If scientists can determine how matter, energy, time, and space behaved during the first nanoseconds of existence, they could potentially resolve the discrepancies between relativity and the Standard Model. This research is being done at the Large Hadron Collider in Switzerland, which smashes particles together at extremely high energies in an attempt to replicate the conditions that existed in the universe’s first moments.)
However, the Standard Model is woefully incomplete. One issue that Kaku is quick to point out is that the Standard Model is an unsightly mess—a chimeric creation of theories stitched together in a way that isn’t completely plausible and contains mathematical terms that aren’t greatly understood. On the plus side, the equations for the Standard Model are symmetrical. But, Kaku says that, in this case, that’s a problem. The universe around us isn’t symmetrical—matter is clumped into stars and galaxies with vast expanses of empty space between them. If the Standard Model were true, the universe would instead be a perfectly uniform sea of particles. Obviously, something is amiss.
For the Standard Model to produce the asymmetrical universe of today, something must have shattered the initial symmetry shortly after the Big Bang occurred. Researchers proposed an undiscovered “Higgs boson” consistent with the Standard Model that unbalanced the cooling universe’s symmetry and led to the variety of particles known today. Sometimes called the “God particle,” the Higgs boson created regions of space within which other particles began to have mass. Kaku writes that, because of this imbalance, the original ruling force split into the strong and weak forces, electromagnetism, and gravity. Confirming the existence of the Higgs boson was one of the missions of the Large Hadron Collider, which it accomplished in 2012.
The Higgs Boson One point that Kaku doesn’t state explicitly is that the Standard Model’s equations are symmetrical only if you presume that subatomic particles have no mass. Once mass is introduced into the equations, their inherent symmetry falls apart and they become enormously complex and contradictory. In 1964, Peter Higgs proposed a solution—an invisible field permeating space that pushes back on particles as they accelerate, imbuing them with what we perceive as mass. Higgs’s suggestion was initially rejected, though other physicists eventually warmed to the idea. If mass is the product of an external “Higgs field,” then it doesn’t have to be accounted for in the Standard Model’s particle equations. However, since the Standard Model explains fields as being produced by carrier particles, such as the photon and the gluon, the Higgs field must have a particle too—the Higgs boson. The Large Hadron Collider (LHC) detected the Higgs boson indirectly by smashing other particles together and interpreting the data from their collisions. As subatomic particles break apart, their components decay into a variety of components, and out of every billion LHC collisions, the collider produced a particle that matched predictions of the Higgs boson’s properties. |
The Gravity Problem
Despite the work done to confirm the Standard Model, it only applies in the realm of quantum mechanics. To achieve a theory unifying all of physics, gravity will have to be folded into the equations for all the other forces. Here, we’ll explain the struggles involved with incorporating gravity into the Standard Model and several discoveries that have been made along the way.
The trouble with gravity is that it’s by far the weakest of the universal forces, so it’s virtually impossible to observe on the quantum scale. While Einstein defines gravity as a curvature in space, there’s no way to express this in the mathematics of quantum mechanics. Instead, physicists have tried another tack—to model gravity as a force created by a particle. Just as gluons transmit the strong nuclear force, scientists have proposed the existence of gravitons that govern gravitational fields. While plausible, Kaku writes that so far no one’s been able to create a mathematical model of gravitons that produces meaningful results.
(Shortform note: Despite Kaku’s admission that gravitons lack a firm mathematical model, researchers are already thinking about ways that they might be detected experimentally. One approach takes advantage of the idea that force-bearing particles like gravitons and photons can, on rare occasions, convert back and forth. By observing a space where graviton concentration would be highest —such as between colliding black holes—astronomers might witness flashes of light as handfuls of gravitons transform into photons. Another approach takes advantage of the Casimir effect, in which metallic plates are attracted to each other in a vacuum if they’re placed near enough to experience the effect of quantum forces.)
Despite the problems of incorporating gravity into quantum mechanics, that hasn’t stopped people from trying. Famed physicist Stephen Hawking has done so by considering what happens in the subatomic world in the one place where gravity can’t be escaped—the event horizon of a black hole, or the dividing line around a black hole’s zone of no return. Black holes are objects so massive and dense that their gravity stops even light from escaping. Kaku says that in this realm of gravitational extremes, Hawking discovered that quantum forces slowly drain energy and mass from a black hole’s gravitational field.
This takes place because, in the subatomic world, empty space isn’t really empty. Kaku points out that in quantum mechanics, empty space is actually a bubbling sea of symmetrical positive and negative particles that pop into being and cancel each other. Normally, these particles vanish before they affect their surroundings, but near a black hole, half of the pair may be sucked in while the other flies free. Since energy can’t be created or destroyed, the energy to create this particle must be subtracted from the black hole, reducing its gravitational pull. In other words, near a black hole, gravity and the quantum world intersect.
Simulating Black Holes in the Lab Dubbed “Hawking radiation,” the quantum energy drain from a black hole that Kaku describes might be nearly impossible to detect. Hawking’s black hole equations show that in addition to being unbelievably massive, black holes are incredibly cold and grow more so as they increase in mass. Black holes “hot” enough to give off perceptible Hawking radiation would have to be smaller than any known to exist. However, scientists have found another way. In 2021, researchers used a Bose-Einstein condensate—a state of matter that only exists when particles are cooled almost to absolute zero—as a way to simulate conditions near a black hole. They detected Hawking radiation from the quantum condensate, which they were able to compare to various models of how Hawking radiation should emerge from black holes. |
Back to the Beginning
Another point in space and time where quantum mechanics and gravity overlap is the moment of the Big Bang itself. Kaku writes that, because of this, physicists are determined to study and model the first fractions of a second of the universe’s existence. In the first instants of time, all the fundamental forces of nature interacted with each other before branching off into their own domains. We study the conditions in those first moments by recreating them in supercolliders and by looking into the farthest depths of space, which—because light takes time to travel—also entails looking backward in time.
Indeed, Kaku affirms that there are several observations about the Big Bang that can only be explained using quantum mechanics. By treating the Big Bang as a source of quantum radiation, researchers were able to compute the temperature of the Big Bang’s leftover heat, which was confirmed when the Cosmic Microwave Background (CMB) was discovered in 1964. Another issue is the universe’s rate of expansion, as measured by the stretched-out light waves detected from distant galaxies, which is much faster than Einstein’s equations predict. Kaku argues that quantum theory accounts for this discrepancy by showing that the universe expanded more rapidly in its early stages than was previously thought.
Physics of the Young Universe The early, rapid expansion that Kaku mentions is referred to as the Inflationary Universe Theory. This theory posits that when the fundamental forces began to diverge in the first few seconds after the Big Bang, they unleashed a surge of energy that made the universe expand even more quickly than before. It’s unclear if this is related to the so-called dark energy that’s currently accelerating the universe’s expansion. Regardless, this early expansion took place 400,000 years before the earliest image of the universe we have—the aforementioned Cosmic Microwave Background. In Astrophysics for People in a Hurry, Neil deGrasse Tyson explains the CMB as a “snapshot” of the early universe at a time when it had cooled enough for photons to pass unimpeded through space without colliding with hot gas particles. Tyson calls attention to the fact that the map of the CMB is lumpy—an uneven mix of hot and cool zones that backs up Kaku’s statement that something unbalanced any symmetry the early universe had. |
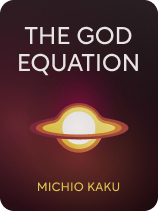
———End of Preview———
Like what you just read? Read the rest of the world's best book summary and analysis of Michio Kaku's "The God Equation" at Shortform.
Here's what you'll find in our full The God Equation summary:
- The search for one theory that would unify all the rules of physics
- Why physicist Michio Kaku thinks string theory may hold the answer
- An explanation of string theory and the underlying symmetries of nature