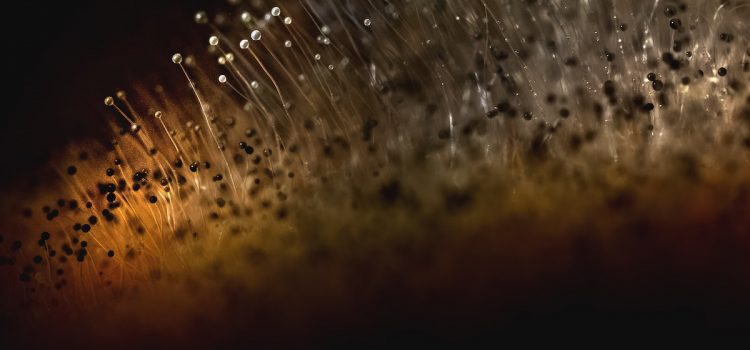
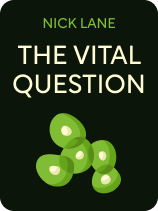
This article is an excerpt from the Shortform book guide to "The Vital Question" by Nick Lane. Shortform has the world's best summaries and analyses of books you should be reading.
Like this article? Sign up for a free trial here.
How did complex life emerge? What distinguishes eukaryotic life from simpler life forms?
In his quest to understand the origin of life, biochemist Nick Lane examines the transition from the simplest life forms (bacteria and archaea) to more advanced organisms (eukaryotes). He explains his research and his resulting proposal in The Vital Question.
Read more to understand Lane’s ideas about the origin of eukaryotes.
The Origin of Eukaryotes
The event that prompted the explosion of complex life from simpler, single-celled forms has long been shrouded in mystery. Lane shares his beliefs about the origin of eukaryotes—the domain of life that includes everything more advanced than bacteria and archaea. He proposes that they arose from a single, unlikely symbiosis between two very different single-celled creatures that unlocked the limits on how cells use energy and undammed the flow of evolutionary progress. Lane explains what distinguishes eukaryotic cells, the idea of evolution by symbiosis, and the energy problem that holds bacteria and archaea back while propelling eukaryotic life to ever greater organization and complexity, eventually evolving into the plant and animal kingdoms.
What defines eukaryotic life is that the cell’s DNA is contained within a protective nucleus, whereas, in archaea and bacteria, the DNA is attached to the outer cell wall right next to the enzymes that produce ATP. Not every eukaryote is multicellular, but they all dwarf bacteria and archaea in terms of the size of their genome and the complexity of their molecular machines. Eukaryotes are full of mitochondria that generate the relatively enormous amount of power eukaryotic cells need to enact the properties of their multitude of genes.
(Shortform note: The single-celled eukaryotes Lane refers to include yeast, algae, and plankton. Yeast is a form of single-celled fungus. But, unlike bacteria, yeast cells have nuclei, mitochondria, and other organelles that simpler life forms lack. The same is true for algae, which has both single-celled and multicellular varieties. Aquatic plankton, which form the base of the oceanic food chain, also fall into this category. Because of the structural complexity of their cells, yeast, algae, and plankton are more closely related to humans than any bacterial species.)
To be sure, bacteria and archaea are incredibly varied in terms of biochemistry, but they don’t come close to eukaryotes in terms of size and complexity. What’s more, bacteria and archaea haven’t evolved cellular complexity in four billion years. Lane suggests that there’s something physically different about eukaryotic cells that allows them the structural complexity that bacteria and archaea can’t achieve. What puzzles biologists is that they’ve found no “missing link” between simple, single-celled organisms and eukaryotes as if the first eukaryotic cell sprang into existence fully formed.
(Shortform note: While Lane discounts proposed “missing link” candidates such as the anaerobic giardia microbes that exist in animals’ intestinal tracts, researchers have offered a new candidate since the time of Lane’s writing. The microbes in question are lokiarchaeota, a member of the Asgard superphylum of archaea that were discovered in vents on the ocean floor between Siberia and Greenland, and later near Japan. These archaea have several genetic and structural similarities to eukaryotes, and while their discovery does not invalidate Lane’s endosymbiosis hypothesis, it does suggest what type of archaea was the original host cell from which eukaryotes sprung.)
The Great Merger
An analysis of eukaryotic genes further confuses the issue of complex life’s evolutionary heritage. Eukaryotes share a third of their genome with single-celled life, but 75% of those genes come from bacteria while the rest come from archaea, as if eukaryotes somehow descended from both domains of simple organisms. Lane says that this is evidence that the first complex cell was a hybrid of a bacterium and an archaeon that somehow grafted themselves together. Because this shared genome is common to all eukaryotes, biologists are forced to conclude that this hybridization only happened once in four billion years, or at least only once that was successful and survived.
(Shortform note: The specific word Lane uses is “chimera” rather than hybrid. In biology, a hybrid is an offspring of parents from two different breeds, subspecies, or species, such as mules, which result from breeding horses and donkeys. A chimera, on the other hand, is a life form that has distinct cells with different sets of genes. Named for the three-headed creature of myth with a body composed of goat, lion, and snake, real-life chimerism has been observed in anglerfish, cats, and even humans. While some chimeras are developed in a lab, they occur in nature when one embryo absorbs another. In humans, chimerism is sometimes unnoticed, though it can also result in mottled skin, mismatched eye colors, and autoimmune disorders.)
The process by which such grafting can occur is called endosymbiosis, in which one cell is physically engulfed by another, but instead of being consumed, the two cells become a larger, combined organism. The evidence that this happened lies in our mitochondria, which have a completely separate genome from the nucleus of the cell. Mitochondria are, in fact, an evolutionary remnant of that first coupling.
Once, long ago, an archaeon absorbed a bacterium in such a way that allowed the bacterium and its descendants to flourish inside the archaeal host cell. Lane explains that the host stripped the bacteria’s DNA, adding it to its own repository, while leaving the bacteria just enough genes to produce ATP and fuel the host cell’s growth. As evidenced by the mitochondrial genome, our modern mitochondria are the descendants of those bacteria, reduced to the status of power batteries that are fed and protected by the larger eukaryotic cell.
Tracking Mitochondrial DNA Across the Ages Lane explores more biological implications about the relationship between nuclear and mitochondrial DNA than can be covered here, but he skips the most commonly known aspect of mitochondrial DNA—that it can be used to trace your maternal ancestry. While the genes in your cells’ nuclei are inherited from both of your parents, your mitochondrial DNA comes solely from your mother. And since historically the human race was far less mobile than it is at present, past mutations in mitochondrial DNA can be tied to diverging ethnic groups as they slowly migrated across the ancient world. Today, there are several mitochondrial DNA testing services you can use for genealogical purposes. Mitochondrial testing is also used as a diagnostic tool for hereditary diseases and in forensic analysis of human remains in which nuclear DNA has otherwise decomposed. Mitochondrial DNA testing has uncovered surprises in people’s family trees, allowed descendants of enslaved people to reconnect with their cultural heritage and even helped to identify the remains of King Richard III. All this is merely a small-scale representation of Lane’s deeper truth—that the mitochondria in all complex life share a single genetic ancestor. |
The Energy Problem
The fact that the initial archaea-bacteria chimera thrived suggests that its odd symbiosis provided the first eukaryotes with an evolutionary advantage. As it was with the original genesis of life and the adaptation that let it leave its ocean-vent nest, the development of complex cellular structures revolved around a new way to harness energy. Lane describes the energy restrictions that have kept bacteria and archaea from achieving complexity for billions of years, while the novel approach that eukaryotes took to overcome that energy barrier not only allowed for greater structure and complexity but actually drove genetic development.
One fundamental difference between eukaryotes and simpler life forms is that because of their mitochondria, eukaryotes have vastly more energy available for use per gene. That energy goes into constructing proteins based on DNA’s genetic designs. Without mitochondria to fuel them, bacteria and archaea have to rely on the ATP their genes produce along their outer cell wall. Lane says this encourages them not to grow bigger because as a bacterial cell’s volume increases, its available energy only grows proportional to its increase in surface area. A large cell is therefore less energy efficient and would be outcompeted by its leaner, meaner cousins.
(Shortform note: While Lane describes energy efficiency as a main driving factor behind the evolution of bacteria, some biologists believe that energy efficiency is the primary evolutionary driver for all life on earth. In this view, efficiency is measured as the ratio between the energy a life form takes in versus the energy it had to expend to obtain it. For instance, to calculate a cheetah’s energy efficiency, you’d divide the amount of energy it obtains from eating a gazelle by how much energy the cheetah expends while hunting. It’s been suggested that humans evolved to walk upright because bipedal motion is more energy-efficient than walking on all fours like our chimpanzee cousins.)
Because of the efficiency problem, Lane argues that there’s no evolutionary reason for archaea and bacteria to grow in size and complexity. By adapting their inner metabolic processes, single-celled organisms have adapted themselves to flourish in every corner of the ecosphere, including the Earth’s most extreme environments. There’s no need for more complexity or larger genome size, so evolution has instead selected for bacteria and archaea that are slimmed down to the basic needs of survival.
(Shortform note: The advantages of efficiency that Lane says guide bacterial evolution have led to some very small creatures indeed. The smallest known bacteria—less than a micron (a millionth of a meter)—in diameter, is mycoplasma genitalium. M. genitalium also has the smallest genome of any living microbe capable of reproducing on its own. M. genitalium inhabits the human urinary system, can be sexually transmitted, and causes a host of medical problems in both men and women. M. genitalium is so lean and efficient, it doesn’t even have a cell wall, which makes it resistant to antibiotics that target the cellular structure of larger bacteria.)
During the endosymbiosis event that launched the beginning of eukaryotic life, the evolutionary pressure for lean, efficient cells got flipped on its head. The newly absorbed mitochondrial bacteria provided far more energy than the archaeal host cell needed. Lane suggests that rather than being drowned with ATP molecules, the host cell had to find something to do with all that excess energy. Its solution came from the extra DNA it absorbed and repurposed from its bacterial symbiotes. The flood of new energy demanded it be spent, and the only way for eukaryotes to survive was to open the possibilities of complex cell structures, charting new frontiers for evolution to explore.
(Shortform note: To understand Lane’s idea that a dramatic increase in cellular energy demanded an increase in size and complexity, consider the problem in terms of economics. The archaeal host cell is like a small town—lean, efficient, and relatively stable. However, the inrush of mitochondrial ATP is like a population explosion within the town’s borders. To survive, the town has to grow into a city by creating new infrastructure, housing, and development so that it can employ its booming population. If it doesn’t, then rampant unemployment leads to poverty, hunger, and disease—exactly what happens inside a cell that doesn’t fully employ its ATP.)
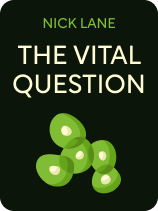
———End of Preview———
Like what you just read? Read the rest of the world's best book summary and analysis of Nick Lane's "The Vital Question" at Shortform.
Here's what you'll find in our full The Vital Question summary:
- Why genetics alone aren't enough to explain why cells function as they do
- A theory on where all complex life originated from
- An explanation of how cells work and are made