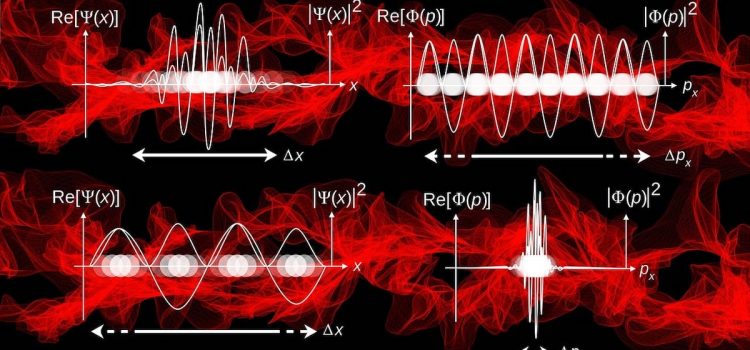
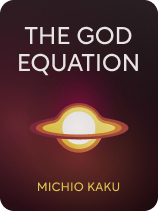
This article is an excerpt from the Shortform book guide to "The God Equation" by Michio Kaku. Shortform has the world's best summaries and analyses of books you should be reading.
Like this article? Sign up for a free trial here.
What surprising things does quantum physics tell us about motion and how matter functions? What’s the most powerful force known in nature?
For centuries, scientists have sought a resolution that some call “the God equation”—a single theory that would unify physics, cancel out its contradictions, and provide one simple, underlying equation from which we can derive a complete understanding of the universe.
According to Michio Kaku, quantum physics makes a vital contribution to this quest. Read more to learn about this fascinating field.
Michio Kaku on Quantum Physics
According to theoretical physicist Michio Kaku, quantum physics is a strange set of rules followed by the laws of nature on the level of the atom. Scientists studying the building blocks of matter discovered quantum physics while Einstein was solving the riddles of gravity and motion.
We’ll trace the road to quantum physics from attempts to explain how objects radiate heat to the discovery that some subatomic particles also behave like waves instead of matter and finally to a unification between the new quantum theory and Maxwell’s light equations.
In the early 1900s, our model of the atom was like a miniature solar system with a tiny, dense nucleus of protons and neutrons surrounded by orbiting electrons. However, theoretical physicist Max Planck noticed something strange about atomic behavior when he investigated the light given off by hot materials. According to equations based on Newton’s laws of motion, the atoms in hot materials vibrate faster than those at cooler temperatures, and they release their excess energy in the form of light. However, the math didn’t add up—the wavelengths of light emitted by hot, glowing matter didn’t match the predictions of Newtonian thermodynamics.
(Shortform note: The building blocks of atoms that are quantum physics’ focus were separately discovered over a 40-year period. In 1897, J. J. Thomson found that negative electrical charges are transmitted by particles tinier than atoms, which he dubbed electrons. In 1911, his student Ernest Rutherford established that atoms have positively charged nuclei, and he coined the term proton in 1920 for the nuclear components that gave it this charge. To account for the total mass of the nucleus, he also proposed an electrically neutral particle, which Rutherford’s student James Chadwick confirmed in 1932 with his discovery of the neutron.)
Kaku writes that Planck found a solution that was just as shocking to the norms of science as Einstein’s theory of relativity. In essence, Planck determined that the energy released by matter as it cools doesn’t diminish on a linear scale but as tiny bundles called “quanta.” It’s as if, when an atom cools, its energy level doesn’t slide down a ramp but instead walks down a flight of stairs where each step is a specific amount of energy. This energy emerges as packets of light—photons—that exactly match the energy of light given off by heated objects.
The Photoelectric Effect Thanks to symmetry, this process that Kaku describes also works in reverse. Just as electrons emit photons of light as they cool to lower energy levels, if you bombard a substance with light, it imbues electrons with extra energy, breaking some loose in what’s known as the photoelectric effect. This effect doesn’t depend on the intensity of the light, but rather on the frequency of the light waves. This doesn’t make sense under the Newtonian model of physics, which dictates that it’s the amount of energy that matters. However, in 1905, while still working on his theory of relativity, Einstein used Planck’s equations to explain the photoelectric effect. He showed that when the energy of photons at specific light frequencies matched electrons’ exact quantum energy levels, that alone was sufficient to knock the electrons into higher quantum energy states and even break them free from their atoms. It was for his explanation of the photoelectric effect that Einstein won the Nobel Prize in Physics, not for his work on relativity. |
Particles and Waves
The problem with photons being particles of light is that Maxwell’s equations show that light is an electromagnetic wave. It turns out that light has properties of both waves and particles, depending on how it interacts with matter. But, Kaku writes that the mystery grew deeper because researchers found that the same was true of electrons. Instead of being envisioned as a particle in orbit around the nucleus, an electron is better described as a wave that wraps the nucleus in a shell. That wave can exist only at specific energy levels that match the quantum orbits in which electrons are found.
(Shortform note: This wavelike twist on our model of the atom is referred to as the electron cloud. While the earlier “solar system” model of the atom presumed that electrons remained at fixed distances from the nucleus, the electron cloud model suggests that electrons can be anywhere around the nucleus—it’s simply much more likely that they’ll be located within specific quantum shells.)
So, when we say that electrons are waves, it simply means that you can’t pin one down—you can only determine the probability of its location at any given point, and that probability flows like a wave. This goes against all our prior conceptions of motion. Imagine if you can’t tell where the moon is in the sky—you can only compute where it’s likely to be. This makes no sense in our macroscopic world governed by laws of relativity, but it’s exactly how electrons and photons, as well as other particles, behave. Clearly, says Kaku, if we’re to uncover one universal theory, it will have to reconcile quantum physics with Einstein’s special relativity.
(Shortform note: While Kaku’s focus is on the subatomic world, there’s a large-scale example of a phenomenon in which we can only make probabilistic predictions—the weather. Meteorology relies on chaos theory, a branch of math that calculates probabilities in systems that have so many uncertain variables that completely accurate predictions are impossible. In the world of subatomic particles, it appears that a degree of uncertainty is baked into the very fabric of the universe—for any phenomenon that behaves like a wave, it’s impossible to measure its exact speed and direction at any given moment in time.)
The Heart of the Atom
The quest to unify relativity and quantum theory is ongoing. In the meantime, scientists pushed quantum physics further so they could unravel the secrets of the atom. To enter the subatomic realm, we’ll explain researchers’ methods to pry open the atom’s nucleus, the discovery of even smaller subatomic particles, and how combining the mathematical models of the forces which interact inside the atom leads to a theory of how all matter functions.
The only way to look inside atoms is to break them apart. Using accelerators, researchers smash atomic nuclei together and study the pieces found in the wreckage. When this was first done, physicists were surprised to find that protons and neutrons are composed of even smaller particles known as quarks. Studies revealed different types of quarks, and that protons, neutrons, and even stranger particles are made from arrangements of groups of three quarks each. Kaku says the equations governing quarks are symmetrical because you can move around any of the three quark components and the particle they create stays the same.
(Shortform note: In addition to the symmetry that Kaku calls attention to, quarks come in six different types, grouped into three symmetrical pairs—“up” and “down” quarks, “top” and “bottom” quarks, “charm” and “strange” quarks—though only up and down quarks make up protons and neutrons. The other quarks are building blocks of strange, exotic particles that can only exist under special conditions, such as the short-lived J/psi meson that proved the existence of charm quarks. Another example is the Lambda baryon—a neutron-like particle containing strange quarks that may be prevalent in superdense neutron stars.)
What holds quarks together into protons and neutrons, and holds neutrons and protons together in the atom, is the most powerful force known in nature—the strong nuclear force. This force is an extremely short-range field of attraction generated by a particle called a gluon (because it glues subatomic particles together). This field can be described mathematically using a modified version of Maxwell’s equations that covers fields of attraction and repulsion beyond that of electromagnetism. Kaku writes that equations such as these that explain different forces are key steps along the path to a unified theory of physics.
(Shortform note: Though Kaku doesn’t pose it this way, at the subatomic level of quantum physics, the forces of nature are actually the result of energy being transferred from one particle to another via smaller particles that act as couriers. The field of the strong force binding the nucleus is the result of gluons zipping back and forth between protons and neutrons, exchanging energy, just as the electromagnetic force binding electrons to their atomic shells is transmitted by the interchange of photons. In this sense, the forces are better described as “interactions” between particles.)
The Weak Interaction
In addition to electromagnetism, which keeps electrons in orbit around the nucleus, and the strong nuclear force, which holds the nucleus together, there is also the “weak interaction,” also known as the weak nuclear force. Whereas the strong force holds atoms together, the weak nuclear force breaks particles apart and can even change them from one form to another.
(Shortform note: Though stronger than gravity, the weak force is less powerful than either the strong force or electromagnetism, and it only operates on the tiniest scale—inside protons and neutrons. The action of the weak force is to transform quarks from one type to another—for example, from top to bottom, or vice versa. This change in state for one quark in a neutron can change the neutron itself into a proton, altering the atomic nucleus of which it is a part. The example of the weak interaction Kaku points to is a form of radiation known as beta decay.)
In beta decay, the weak force breaks a neutron apart into a proton, an electron, and the electron’s phantom partner, the neutrino. Neutrinos have no electric charge and virtually no mass. As such, a neutrino can pass through stars and planets without stopping. Nevertheless, Kaku says the neutrino fills a key gap in the atomic model that was predicted by symmetry—if the proton has an electrically neutral counterpart (the neutron) shouldn’t the electron have one too? Their existence was purely theoretical until they were observed in 1956.
(Shortform note: Kaku doesn’t explain how scientists were able to capture neutrinos if they’re so elusive. In the 1950s, researchers devised an experiment by placing light-emitting material inside a giant water tank positioned near a nuclear reactor. The nuclear plant would theoretically produce trillions of neutrinos per second, an infinitesimally small fraction of which would interact with the matter in the tank, making it glow. In order to detect the light from neutrinos, the tank had to be shielded from every other form of radiation, including cosmic rays from outer space. Even so, it took more than five months of observations to confirm that neutrinos actually exist.)
Once neutrinos were confirmed to exist, they gave insights into the weak nuclear force that let physicists unite it with the electromagnetic force. Today it’s understood that electromagnetism and the weak nuclear force are one and the same, now referred to as the electroweak force. Kaku affirms that this is the path that the search for a unifying theory has taken—merging separate theories about phenomena until there’s only one left.
(Shortform note: Merging the weak and electromagnetic forces isn’t something that’s easily achieved. While Kaku hints at how it’s done mathematically, in practice the two forces only act the same at temperatures that haven’t existed since the first picosecond after the Big Bang. The first experimental evidence for the existence of the electroweak force was discovered in 1973 at the European Council for Nuclear Research (CERN) neutrino detector in Switzerland, followed by the discovery of the particles that transmit the electroweak force in 1983 using CERN’s Super Proton Synchrotron.)
Exercise: Reflect on Quantum Physics
At the subatomic level of quantum physics, particles obey completely different rules than those we experience in our day-to-day lives. Scientists conclude from this that our understanding of physics must be flawed because two different sets of physical laws can’t both be true at the same time. What do you think about this apparent contradiction?
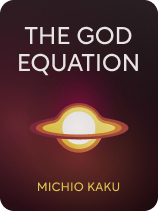
———End of Preview———
Like what you just read? Read the rest of the world's best book summary and analysis of Michio Kaku's "The God Equation" at Shortform.
Here's what you'll find in our full The God Equation summary:
- The search for one theory that would unify all the rules of physics
- Why physicist Michio Kaku thinks string theory may hold the answer
- An explanation of string theory and the underlying symmetries of nature